Quantitative Polymerase Chain Reaction (qPCR), also known as real-time PCR, stands as a cornerstone in the field of molecular biology, revolutionizing the way researchers quantify nucleic acids. This powerful technique combines the principles of traditional PCR amplification with the ability to monitor the process in real time, allowing for the precise quantification of DNA or RNA present in a sample.
qPCR offers unparalleled precision and efficiency, making it an invaluable tool across various scientific disciplines. Its ability to detect and quantify minute amounts of target genetic material enables applications ranging from medical diagnostics and infectious disease research to environmental monitoring and forensic analysis. By providing real-time data collection, qPCR offers immediate insights into the amplification of nucleic acids, which is pivotal for studies requiring accurate quantification and dynamic response measurements. This method not only enhances the robustness of experimental outcomes but also significantly accelerates the research process by delivering faster results compared to end-point PCR techniques.
In this guide, we’ll explore the fundamental aspects of qPCR, delve into its operational mechanics, and discuss its broad range of applications, ensuring that readers gain a comprehensive understanding of this indispensable molecular biology technique.
Basic Principles of qPCR
Definition and Fundamental Concepts of qPCR
Quantitative PCR (qPCR), often referred to as real-time PCR, is a technique used to amplify and quantitatively measure DNA sequences in a sample. Unlike traditional PCR, which only provides data at the end of the process, qPCR monitors the amplification of DNA during each cycle of the PCR in real time. This is achieved through the use of fluorescent markers that emit light proportional to the amount of PCR product formed during the reaction.
The core principle of qPCR is based on the detection of fluorescence emitted by a reporter molecule, which increases as the PCR product accumulates. There are two main types of reporter mechanisms:
- Dye-based reporters: These bind to any double-stranded DNA, increasing fluorescence with each DNA doubling. SYBR Green is a common example of a dye used in qPCR.
- Probe-based reporters: These involve sequence-specific oligonucleotide probes labeled with a fluorescent reporter, which permits the detection only when the probe hybridizes to its complementary DNA target, providing enhanced specificity.
How Probe-Based Reporters Work
- Design and Structure: Probe-based reporters consist of short, single-stranded DNA or RNA molecules that are specifically engineered to be complementary to a sequence within the target DNA. Each probe is labeled with two crucial components:
- Fluorescent Reporter Dye: This dye emits fluorescence when excited by a light source, but its emission is initially quenched due to the proximity of a quencher molecule on the same probe.
- Quencher Molecule: Positioned near the fluorescent dye, the quencher absorbs the fluorescence emitted by the dye as long as the probe remains intact.
- Hybridization During PCR: During the annealing phase of PCR, the probe binds to its specific target sequence if present in the sample. This hybridization is critical for the functioning of the probe.
- Cleavage in Extension Phase: In the extension phase of PCR, the DNA polymerase extends the primer and begins synthesizing the new DNA strand. As the polymerase encounters the bound probe, its 5′ to 3′ exonuclease activity cleaves the probe. This cleavage separates the fluorescent dye from the quencher.
- Fluorescence Emission: Once the fluorescent reporter dye is separated from the quencher due to the cleavage of the probe, it starts emitting fluorescence upon excitation. The intensity of this fluorescence is proportional to the amount of target DNA, as more probe cleavage occurs with more target DNA present.
Advantages of Probe-Based Reporters
- Enhanced Specificity: The need for probe hybridization to the specific target sequence greatly reduces the chances of non-specific amplification, which can be a problem with dye-based methods like SYBR Green that bind any double-stranded DNA.
- Reduced Background Noise: Since the fluorescence is only emitted upon probe cleavage and hybridization to the target, background fluorescence is minimal, enhancing the dynamic range and sensitivity of detection.
- Multiplexing Capability: Different probes can be labeled with different fluorescent dyes, allowing for the simultaneous detection of multiple targets in a single PCR reaction. This is particularly useful in diagnostic applications where multiple pathogens need to be screened in one go.
- Quantitative Accuracy: The direct correlation between fluorescence emission and the amount of target DNA provides precise quantitative data, crucial for applications such as viral load determination or gene expression analysis.
Probe-based qPCR is thus a powerful method for accurate, specific, and sensitive quantitative PCR. It is widely used in clinical diagnostics, genetic research, and any application where precision and reliability are paramount.
Key Components of qPCR
Quantitative PCR (qPCR) relies on several critical components that work together to enable the precise amplification and quantification of nucleic acids. Understanding these components is essential for conducting effective qPCR experiments. Here’s an overview of the main elements involved in qPCR and the various fluorescence chemistries that are employed.
Main Components of qPCR
- Thermal Cyclers: The fundamental device used in qPCR is a thermal cycler equipped with fluorescence detection capabilities. Thermal cyclers are designed to rapidly change temperatures in precise and controlled steps, which is crucial for the different stages of PCR: denaturation, annealing, and extension. Modern thermal cyclers for qPCR also integrate real-time fluorescence measurement to monitor the amplification process as it occurs.
- Fluorescence Detection Systems: These systems are integrated into the thermal cycler and are responsible for exciting the fluorescent markers used in the qPCR and detecting the emitted light. The data collected from the fluorescence signals is used to calculate the quantity of the target nucleic acid in real time. These systems must be highly sensitive and capable of detecting changes in fluorescence across a range of concentrations and through numerous PCR cycles.
- Optical Components: Part of the fluorescence detection system, optical components include filters and lenses that allow for the specific excitation and detection of fluorescence emitted from the dyes or probes bound to the DNA. The optical system must be capable of distinguishing between different dyes when multiplex qPCR is performed.
Fluorescence Chemistries Used in qPCR
Fluorescence chemistries in qPCR are critical for enabling the detection and quantification of DNA. There are primarily two types of fluorescence chemistries used:
- SYBR Green I Dye: This is a double-stranded DNA-binding dye that fluoresces upon binding to any double-stranded DNA. During qPCR, as the DNA template is amplified, more dye binds to the newly formed double-stranded DNA, increasing the fluorescence intensity. SYBR Green is advantageous due to its simplicity and cost-effectiveness. However, its non-specific binding nature requires careful design and validation of primers to minimize the amplification of non-target sequences.
- TaqMan Probes: These are fluorophore-labeled sequence-specific probes that increase fluorescence only when hybridized to their complementary target sequence. As the PCR progresses and more target DNA is amplified, more probes are cleaved by the DNA polymerase’s exonuclease activity, separating the fluorophore from the quencher and increasing fluorescence. TaqMan probes offer higher specificity compared to SYBR Green and are particularly useful in applications requiring precise quantification and multiplexing capabilities.
Functionality of Fluorescence Detection Systems in qPCR
- Excitation of Fluorescent Markers: The system uses a light source, typically a laser or a high-intensity LED, to emit light at specific wavelengths that excite the fluorescent markers used in the qPCR reactions. These markers could be intercalating dyes like SYBR Green or sequence-specific probes such as TaqMan probes.
- Detection of Emitted Light: Once the fluorescent markers are excited, they emit light at a different wavelength. This emitted light is captured by the detector in the thermal cycler. The detectors are typically photodiodes or charge-coupled devices (CCDs) that are sensitive enough to detect even low levels of fluorescence.
- Filtering System: To accurately capture fluorescence, the detection system includes optical filters that permit only the wavelengths emitted by the fluorescent markers to pass through, thus enhancing the specificity of detection and reducing background noise.
- Real-Time Monitoring: During each cycle of the qPCR, the fluorescence detection system measures the intensity of the fluorescence after the extension phase. This measurement is crucial as it indicates the amount of PCR product generated in each cycle, providing real-time insights into the amplification process.
- Data Analysis and Quantification: The real magic of fluorescence detection systems lies in their ability to convert the detected fluorescence into quantitative data. Software integrated with the thermal cycler analyzes the fluorescence data to calculate the initial quantity of the target nucleic acid. This analysis is often displayed in the form of amplification plots that show the increase in fluorescence over PCR cycles, reflecting the exponential amplification of the target DNA.
Importance and Sensitivity
The sensitivity of the fluorescence detection system is pivotal for the success of qPCR. These systems must accurately detect changes in fluorescence across a wide range of target concentrations and throughout numerous PCR cycles. This high sensitivity ensures that even small amounts of nucleic acid in a sample can be detected and quantified accurately, which is especially important for clinical diagnostics, environmental biology, and other fields where precise measurements are critical. The ability to detect slight variations in fluorescence also allows for the early determination of the amplification kinetics, which can provide insights into the efficiency and specificity of the PCR reaction, helping researchers to optimize conditions and interpret results accurately.
Data Analysis and Quantification in qPCR
The effectiveness of quantitative PCR (qPCR) is largely dependent on the precision and capabilities of data analysis and quantification. This phase is where the real-time measurements of fluorescence are translated into valuable data regarding the quantity of nucleic acids in a sample. Here’s how this crucial part of the qPCR process works:
Conversion of Fluorescence to Quantitative Data
- Fluorescence Measurement: During each cycle of the qPCR, the fluorescence detection system measures the fluorescence intensity emitted from the sample. This intensity increases as more PCR product is generated, provided that the fluorescent markers are properly binding.
- Software Analysis: The thermal cycler is equipped with sophisticated software that captures and analyzes these fluorescence signals. This software is designed to manage the complex data and perform statistical analyses to interpret the fluorescence measurements accurately.
Calculating Initial Nucleic Acid Quantities
- Baseline and Threshold Setting: The software first establishes a baseline fluorescence, which is the level of fluorescence inherent to the system or the background noise. It then sets a threshold level, which is minimal enough to detect significant changes but high enough to avoid background noise.
- Threshold Cycle (Ct): The key to quantification in qPCR is determining the cycle threshold (Ct), which is the cycle number at which the fluorescence surpasses the threshold level, indicating a detectable level of amplified product. The Ct value is inversely proportional to the amount of target nucleic acid in the sample; lower Ct values indicate higher initial quantities of target nucleic acid.
Amplification Plots and Data Interpretation
- Amplification Plots: The software generates amplification plots that graph the increase in fluorescence over each cycle of the PCR. These plots are crucial for visualizing the amplification kinetics and determining the efficiency of the PCR reaction.
- Efficiency Calculation: From the amplification plots, the software can calculate the efficiency of the PCR reaction based on the slope of the fluorescence increase. Ideal reactions should double the amount of DNA with each cycle, reflecting in a steep and linear phase of the amplification plot.
- Quantitative Comparisons: For comparative studies, such as gene expression analysis, relative quantification can be performed by comparing the Ct values of target genes to those of reference genes. This method, often referred to as ΔΔCt method, allows for normalization and comparison across different samples or experimental conditions.
Importance of Data Analysis in qPCR
The data analysis phase in qPCR is critical because it provides the quantitative aspect of the PCR that traditional methods do not offer. By accurately quantifying the amount of initial target nucleic acid, researchers can perform detailed gene expression studies, pathogen quantification, genetic variation analysis, and more. The precision and reliability of this data are paramount, as they can influence the interpretation of biological processes and the direction of future research. In summary, the integration of advanced fluorescence detection systems with powerful analysis software enables qPCR to deliver precise and actionable quantitative data, making it a fundamental technique in molecular biology and a variety of other scientific disciplines.
Software Analysis in qPCR
The efficiency and accuracy of quantitative PCR (qPCR) depend significantly on the software that analyzes the fluorescence data collected during the PCR cycles. This software is integral to the thermal cycler, functioning as the computational and analytical brain of the operation. Here’s a detailed look at how software analysis plays a crucial role in qPCR:
Key Functions of qPCR Software
- Data Capture: The software is responsible for capturing real-time fluorescence data emitted during each cycle of the qPCR. This involves recording the intensity of fluorescence at specific intervals, which corresponds to the progression of DNA amplification.
- Signal Processing: After data capture, the software processes these signals to filter out background noise and enhance the signal-to-noise ratio. This step is critical for ensuring that the readings reflect true DNA amplification and not artifacts or anomalies.
- Baseline Establishment: One of the initial tasks of the software is to determine the baseline fluorescence, which is the level of signal detected before significant amplification occurs. This baseline is used to differentiate between the background fluorescence and the true signal from the target DNA.
- Threshold Setting: The software sets a threshold level, which is critical for determining the point at which the fluorescence is considered significant enough to indicate DNA amplification. The cycle at which the fluorescence exceeds this threshold is known as the threshold cycle (Ct), and it is crucial for quantifying the DNA.
Statistical Analysis and Interpretation
- Ct Calculation: The software calculates the Ct value for each sample, which is the cycle number at which the fluorescence surpasses the threshold. This value is inversely proportional to the amount of target DNA initially present in the sample.
- Amplification Efficiency: By analyzing the shape and slope of the amplification curve, the software can assess the efficiency of the PCR. Efficient reactions should show a consistent and exponential increase in fluorescence corresponding to the doubling of the DNA with each cycle.
- Quantitative Analysis: For quantitative studies, the software can compare Ct values across samples to determine relative amounts of the target nucleic acid. This is often used in gene expression studies where the expression levels of genes are compared under different experimental conditions.
- Normalization and Comparative Analysis: In cases where multiple targets or reference genes are used, the software performs normalization to account for variability in sample loading or reaction efficiency. This normalized data is used for comparative analyses, providing more accurate and reliable results.
Importance of Software in qPCR
The sophisticated software used in qPCR thermal cyclers is what transforms the raw fluorescence data into meaningful, quantitative information. Without robust software analysis, the precision and utility of qPCR would be greatly diminished. The ability to accurately and efficiently process complex data sets allows researchers to perform detailed biological and medical analyses, making qPCR a powerful tool in modern scientific research. In summary, the software analysis component of qPCR thermal cyclers is essential for interpreting the fluorescence signals accurately, calculating quantitative data, and ensuring the reliability and repeatability of the qPCR assays. This software not only supports the basic functions of qPCR but also enhances its applications across various fields of study.
Experimental Design and Setup for qPCR
Designing and setting up a quantitative PCR (qPCR) experiment involves meticulous planning and attention to detail to ensure that the resulting data are reliable and reproducible. Here’s a step-by-step guide covering the critical considerations and the necessary steps to set up a successful qPCR assay.
Critical Considerations in Designing a qPCR Experiment
- Objective Clarity:
- Clearly define the purpose of the experiment. Whether it’s gene expression analysis, mutation detection, or pathogen quantification, the objective will guide all other aspects of the experimental design.
- Selection of Target and Reference Genes:
- Choose appropriate target genes based on the study’s aim. For gene expression studies, select genes relevant to the biological processes or pathways of interest.
- Identify stable reference genes for normalization. These should be genes with consistent expression levels across all samples and conditions in the experiment.
- Primer and Probe Design:
- Design or select primers and probes that are specific to the target sequence. Avoid regions with high secondary structure or sequence similarity to other DNA sequences in the genome.
- For probe-based assays, ensure the probe is labeled with a suitable fluorophore and quencher.
- Sample Quality and Preparation:
- Use high-quality RNA or DNA, free from contaminants that might inhibit PCR. The integrity of nucleic acids should be verified, e.g., via gel electrophoresis for RNA.
- Consider the method of sample preservation and preparation, as these can affect the quality of the nucleic acids.
- Controls and Replicates:
- Include appropriate controls such as no-template controls (NTC) to check for contamination, and positive controls to validate the reaction setup.
- Plan for technical replicates to assess the precision of the qPCR assay and biological replicates to account for natural variations among samples.
Steps to Set Up a qPCR Assay
- Sample Collection and Preparation:
- Collect samples under consistent conditions and process them promptly to minimize degradation of nucleic acids.
- Extract RNA or DNA using a method that suits the sample type and ensures high purity and integrity.
- cDNA Synthesis (for RNA targets):
- For RNA-based applications, synthesize cDNA using reverse transcription. Choose between one-step or two-step RT-qPCR based on the needs of your experiment.
- Setting Up the qPCR Reaction:
- Prepare the qPCR mix, including the DNA template, primers, probes (if applicable), qPCR master mix (which includes DNA polymerase, dNTPs, MgCl2, and buffer), and any other required additives.
- Pipette the reaction mixture into the wells of a qPCR plate, ensuring no cross-contamination occurs.
- Running the qPCR Cycle:
- Program the thermal cycler according to the optimal conditions for your primers and probes. This typically includes an initial denaturation step, followed by 40 cycles of denaturation, annealing, and extension.
- Set up the machine’s software to detect and record the fluorescence data at the appropriate stages.
- Data Collection and Analysis:
- Monitor the amplification in real-time through the software associated with the qPCR instrument.
- Analyze the data post-amplification to interpret the results based on the amplification curves and Ct values.
Conclusion
A well-designed qPCR experiment not only involves choosing the right components and following precise steps but also demands an understanding of the biological variables and technical limitations of the assay. With careful planning and execution, qPCR can provide powerful insights into genetic expression, regulation, and more, making it an indispensable tool in molecular biology research.
Setting Up the qPCR Reaction
Proper preparation of the qPCR reaction mix and accurate pipetting are crucial for the success of a quantitative PCR (qPCR) experiment. Here’s a detailed look at the steps involved in setting up the qPCR reaction:
Preparation of the qPCR Mix
- Components of the qPCR Mix:
- DNA Template: The amount of template added to each reaction should be carefully quantified to ensure consistent and reproducible results. The template DNA should be free of contaminants that might inhibit the PCR.
- Primers: Add forward and reverse primers at optimized concentrations to ensure efficient and specific amplification of the target sequence.
- Probes: If using a probe-based detection method (such as TaqMan probes), include a fluorophore-labeled probe specific to the target sequence. The probe enhances the specificity of the detection.
- qPCR Master Mix: This commercially available mix typically includes Taq DNA polymerase, dNTPs (deoxynucleotide triphosphates), MgCl2, and reaction buffer. The master mix may also contain enhancers and stabilizers that improve the efficiency and specificity of the reaction.
- Other Additives: Depending on the specific requirements of the experiment, additives such as ROX passive reference dye or other enhancing agents might be included to stabilize the reaction or compensate for fluorescence fluctuations.
- Mixing the Components:
- Thoroughly mix the components to ensure a homogeneous reaction mixture. Vortex the mix gently and spin down briefly in a microcentrifuge to collect all contents at the bottom of the tube.
Pipetting the Reaction Mixture
- Loading the qPCR Plate:
- Carefully pipette the reaction mixture into the designated wells of a qPCR plate. The volume typically ranges from 10 to 25 μL per well, depending on the protocol and the plate format.
- Use a fresh pipette tip for each sample to avoid cross-contamination between samples. Cross-contamination can lead to false positives and affect the accuracy of the results.
- Avoiding Air Bubbles:
- Ensure that no air bubbles are introduced while pipetting, as bubbles can interfere with the fluorescence measurements during the qPCR. If bubbles are formed, gently tap the plate or use a needle to burst the bubbles.
- Sealing the Plate:
- Seal the plate with an optical adhesive cover or a lid that is compatible with the qPCR instrument. This sealing prevents evaporation during the thermal cycling process and maintains the integrity of the samples.
- Loading the Plate into the Thermal Cycler:
- Place the sealed plate into the thermal cycler, ensuring it is properly aligned and secured in the instrument. Incorrect placement can lead to uneven heating or issues with fluorescence detection.
Running the qPCR Cycle
Executing the qPCR cycle accurately is essential for the successful amplification and quantification of DNA in a quantitative PCR assay. The process involves precisely programming the thermal cycler and setting up the system to capture the necessary data at the right moments. Here’s a detailed guide on how to run the qPCR cycle effectively:
Programming the Thermal Cycler
- Setting the Cycling Parameters:
- Initial Denaturation: Start with an initial denaturation step, typically at 95°C for about 10 minutes. This step is crucial as it ensures that the DNA template is fully denatured, i.e., the double-stranded DNA is separated into single strands, allowing the primers to anneal in subsequent steps.
- Denaturation Phase: Program the cycler for denaturation at 95°C for 15 seconds for each cycle. This step ensures that the DNA template strands separate before each round of synthesis.
- Annealing Phase: Set the annealing temperature based on the melting temperatures (Tm) of the primers. This typically ranges from 55°C to 65°C and lasts about 20-30 seconds. During annealing, the primers bind to their complementary sequences on the single-stranded DNA template.
- Extension Phase: Program the extension phase at 72°C for about 30 seconds (longer for larger DNA fragments). During this phase, the DNA polymerase synthesizes the new DNA strand by adding dNTPs to the 3′ end of the primed sequence.
- Number of Cycles:
- A typical qPCR includes 40 cycles, allowing exponential amplification of the target DNA. Each cycle theoretically doubles the amount of DNA, leading to a significant accumulation of the PCR product that can be quantified.
Setting Up the Detection System
- Fluorescence Detection:
- Configure the software to measure the fluorescence intensity at the end of each extension phase. This timing is crucial as it corresponds to the maximum amount of double-stranded DNA in the reaction, which binds to the fluorescent markers.
- Ensure that the system is calibrated to detect fluorescence from the specific dyes or probes used in your assay. This may involve setting up filters and sensitivity settings appropriate for the fluorophores involved.
- Real-Time Data Capture:
- The qPCR software should be set to capture and record the fluorescence data in real-time during each cycle. This data is used to generate amplification plots that show the increase in fluorescence over the course of the PCR cycles.
- Ensure that the baseline and threshold settings are adjusted correctly in the software. The baseline settings account for the initial few cycles where fluorescence is negligible, and the threshold setting is used to determine the cycle threshold (Ct) values.
Monitoring and Adjustments
- Monitor the Reaction: Throughout the qPCR run, monitor the amplification plots and other parameters provided by the thermal cycler’s software to ensure that the reaction is progressing as expected.
- Make Adjustments if Necessary: If unexpected results or issues are observed (e.g., amplification occurring too early or too late, or not at all), adjustments might be needed in primer concentrations, annealing temperatures, or the cycling parameters.
Chemistry and Reagents in qPCR
Quantitative PCR (qPCR) utilizes specific chemistries and reagents to enable the detection and quantification of nucleic acids. The choice of chemistry significantly influences the specificity, sensitivity, and applicability of the qPCR assay. Here, we’ll explore the most commonly used qPCR chemistries—SYBR Green and probe-based systems (such as TaqMan)—and discuss the selection of dyes and quenchers that are critical for optimal performance.
SYBR Green Chemistry
Advantages:
- Cost-Effectiveness: SYBR Green is generally less expensive than probe-based systems, making it a cost-effective option for many laboratories.
- Simplicity: This dye binds to any double-stranded DNA, which simplifies the design process as specific probes are not required.
- Flexibility: SYBR Green can be used for a wide range of applications, including gene expression analysis, DNA quantification, and melting curve analysis (which can distinguish between different amplicons based on their melting temperatures).
Considerations:
- Specificity: Since SYBR Green binds to any double-stranded DNA, it may also bind to non-specific PCR products such as primer-dimers or off-target amplifications. This can lead to false positives if not carefully controlled.
- Optimization: It requires careful primer design and optimization of PCR conditions to minimize the formation of non-specific products.
- Post-Amplification Analysis: Melting curve analysis is necessary after amplification to confirm the specificity of the PCR products.
Probe-Based Systems (TaqMan)
Specificity:
- High Specificity: TaqMan probes are designed to hybridize specifically to the target sequence, which significantly reduces the likelihood of detecting non-specific products.
- Mechanism: These probes are labeled with a fluorescent reporter dye on one end and a quencher on the other. During PCR, the 5′ to 3′ exonuclease activity of the Taq polymerase cleaves the probe if it is hybridized to the target, separating the dye from the quencher and resulting in fluorescence.
Applications:
- Quantitative Gene Expression: Particularly useful in applications where precise quantification is crucial, such as in diagnostic assays or quantitative gene expression analysis.
- Multiplexing: Different probes labeled with different fluorophores allow for the simultaneous detection of multiple targets in a single reaction, which is advantageous in clinical diagnostics and genetic testing.
Selection of Dyes and Quenchers:
- Fluorescent Dyes: The choice of fluorescent dye depends on the specific requirements of the assay and the capabilities of the qPCR instrument (e.g., FAM, VIC, CY5).
- Quenchers: The selection of a quencher is critical for reducing background fluorescence. Common quenchers include TAMRA and BHQ (Black Hole Quencher), which absorb the energy from the fluorescent dye without re-emitting it as light.
Optimal Performance
- Compatibility with qPCR Instrument: Ensure that the dyes and quenchers chosen are compatible with the detection channels and filters of the qPCR instrument being used.
- Probe Design: Probes must be designed to hybridize within regions of the gene that are unique and conserved, avoiding regions with secondary structures or significant sequence variation.
- Concentration Optimization: The concentrations of primers and probes must be optimized to achieve the best balance between sensitivity and specificity.
Conclusion
The choice between SYBR Green and TaqMan or other probe-based chemistries depends on several factors, including the nature of the experiment, the need for specificity, the availability of resources, and the capacity for multiplexing. Each chemistry has its advantages and limitations, and the selection of appropriate dyes and quenchers plays a crucial role in ensuring the success of a qPCR assay.
Primer and Probe Design in qPCR
Designing effective primers and probes is crucial for the success of a quantitative PCR (qPCR) experiment. The specificity and efficiency of these oligonucleotides directly influence the accuracy, sensitivity, and reliability of the assay. Here, we’ll explore the guidelines for designing primers and probes and discuss why their specificity and efficiency are vital.
Guidelines for Designing Effective Primers and Probes
- Primer Length:
- Typically, primers should be 18-24 nucleotides in length. This length helps ensure adequate specificity while maintaining an optimal melting temperature (Tm) around 60°C.
- Melting Temperature (Tm):
- Primers should have a Tm close to each other to ensure that they anneal to the template at the same temperature. A Tm discrepancy of more than 2°C between paired primers should be avoided.
- GC Content:
- Aim for a GC content of 40-60%. This range helps achieve a stable duplex with the template DNA while avoiding structures like G-quadruplexes or excessive secondary structure that can impede the PCR.
- Avoid Complementarity:
- Ensure that primers do not have complementary regions, especially at the 3′ ends, to prevent the formation of primer dimers.
- Probe Design:
- For TaqMan probes, the probe should ideally be 20-30 nucleotides in length and have a Tm about 5-10°C higher than the primers. This helps ensure stable hybridization during the annealing phase.
- Place the probe on the same strand and within the region amplified by the primers.
- Positioning:
- Avoid placing primers or probes in regions with known polymorphisms or in highly conserved regions when specificity to a particular strain or species is required.
- End Modification:
- Probes typically have a reporter dye on the 5′ end and a quencher on the 3′ end. Ensure these modifications do not interfere with the overall stability and hybridization efficiency of the probe.
Importance of Specificity and Efficiency
- Specificity:
- High specificity in primer and probe design ensures that the amplification is only from the target sequence and not from non-target sequences or potential contaminants. This is particularly crucial in diagnostics and when quantifying low-abundance targets.
- Specificity reduces background noise and increases the dynamic range of the qPCR assay, allowing for more precise quantification.
- Efficiency:
- Efficient primers and probes are designed to hybridize and extend rapidly and completely with each PCR cycle. High efficiency ensures that the amplification is exponential, which is critical for accurate quantification in qPCR.
- Efficient primer and probe design minimizes the cycle threshold (Ct) variability between replicates and across different experimental setups, enhancing the reproducibility of the assay.
Conclusion
The design of primers and probes is a foundational element in setting up a qPCR assay. By adhering to best practices in oligonucleotide design, researchers can significantly improve the performance of their qPCR assays, leading to robust, reliable, and accurate results. These design principles ensure that the qPCR assay will be sensitive enough to detect target nucleic acids even at low concentrations and specific enough to distinguish between closely related sequences, making it an invaluable tool in research and diagnostic contexts.
Reverse Transcription qPCR (RT-qPCR)
Reverse Transcription Quantitative PCR (RT-qPCR) is a powerful molecular technique used to quantify RNA levels in a sample. This method combines reverse transcription (RT) of RNA into complementary DNA (cDNA) followed by quantitative PCR (qPCR) to amplify and quantify the cDNA. Here’s an overview of RT-qPCR, its applications, and a comparison between the one-step and two-step RT-qPCR processes.
Explanation of RT-qPCR
RT-qPCR is primarily used to measure the amount of specific RNA molecules, typically mRNA, in biological samples. This is particularly useful for studying gene expression levels across different conditions or treatments. The process starts with the extraction of RNA from a sample, which is then reverse-transcribed into cDNA. The cDNA serves as the template for subsequent qPCR amplification and quantification.
Applications of RT-qPCR
- Gene Expression Analysis: RT-qPCR is a standard method for quantifying changes in gene expression under various experimental conditions, such as during disease progression, in response to treatments, or environmental changes.
- Viral Quantification: Used extensively in clinical virology to measure viral RNA loads in patient samples, which is crucial for diagnosis and monitoring of viral infections.
- Transcriptomics Studies: RT-qPCR can validate results obtained from broader transcriptomics studies like RNA sequencing, providing a targeted approach to quantify specific transcripts of interest.
- GMO Testing: Detecting and quantifying genetically modified organisms (GMOs) in food and agricultural products.
One-step vs. Two-step RT-qPCR
Both one-step and two-step RT-qPCR are used to measure RNA concentration, but they differ in methodology and application suitability:
- One-step RT-qPCR
- Process: In one-step RT-qPCR, the reverse transcription and the qPCR amplification are conducted in the same reaction tube without opening the tube between the steps. Specific enzyme mixes are used that can handle both reverse transcription and DNA amplification.
- Advantages: Reduced risk of contamination due to fewer handling steps and a closed system; faster overall process as it requires fewer steps.
- Disadvantages: Less flexibility in optimizing the conditions for reverse transcription and PCR independently; potential for reduced efficiency if the optimal conditions for RT and qPCR differ significantly.
- Best Uses: Ideal for high-throughput screenings where speed and throughput are more critical than fine-tuning individual reactions, or when handling highly infectious materials where contamination risk must be minimized.
- Two-step RT-qPCR
- Process: The reverse transcription and qPCR are performed in separate reactions. First, RNA is reverse transcribed into cDNA in one tube. Then, specific aliquots of this cDNA are transferred to a new tube for qPCR amplification.
- Advantages: Allows optimization of each step independently for maximum efficiency and sensitivity; enables multiple qPCR reactions from a single cDNA synthesis reaction, useful for examining multiple targets from the same RNA sample.
- Disadvantages: Higher risk of contamination due to more handling steps; more time-consuming due to the separate steps.
- Best Uses: Preferred when studying multiple genes from the same RNA sample or when precise control over reaction conditions is needed to optimize results.
Conclusion
RT-qPCR remains a cornerstone technique in molecular biology, offering precise quantification of RNA molecules. The choice between one-step and two-step RT-qPCR depends largely on the specific requirements of the experiment, including the need for throughput, flexibility, and sensitivity. Understanding these methods and their appropriate applications can significantly enhance research outcomes in genetics, clinical diagnostics, and beyond.
Multiplexing in qPCR
Multiplexing in qPCR is a technique that allows the simultaneous amplification and quantification of multiple target DNA sequences in a single qPCR reaction. This approach can significantly enhance the efficiency and throughput of genetic analysis, making it a valuable tool in various fields such as diagnostics, research, and forensic science.
Advantages of Multiplexing
- Increased Efficiency: By analyzing multiple targets in a single reaction, multiplexing saves time and reduces the cost per analysis when compared to running separate qPCR reactions for each target.
- Reduced Sample Consumption: Multiplexing is particularly beneficial when sample material is limited, as it maximizes the amount of information that can be obtained from a small amount of starting material.
- Consistency Across Targets: Running multiple targets in the same reaction under identical conditions can enhance the consistency of the results, minimizing variability that might occur due to differences in reaction setup or cycling conditions.
- Enhanced Data Throughput: Multiplex qPCR allows for the simultaneous screening of multiple genetic markers, speeding up data collection and analysis, crucial in high-throughput environments such as clinical diagnostics and microbial pathogen typing.
Considerations and Challenges in Multiplex Assay Design
- Primer and Probe Design:
- Specificity: Each primer and probe set must specifically bind to their respective target without cross-reacting with other primer-probe sets in the mix.
- Optimal Concentration: Primer and probe concentrations must be carefully optimized to ensure efficient and balanced amplification of all targets. Imbalances can lead to preferential amplification of one target over others.
- Fluorescent Labeling:
- Dye Selection: Choose fluorescent dyes with distinct emission spectra to avoid overlap in the signals detected from each target. This is crucial to ensure that the fluorescence signal from one dye does not interfere with the detection of another.
- Quencher Efficiency: Efficient quenchers are essential to prevent background fluorescence and increase the assay’s sensitivity.
- Reaction Conditions:
- Thermal Profile Optimization: The thermal cycling conditions must suit the annealing temperatures of all primer sets used. This might require compromises or adjustments to find a condition that works well for all targets.
- Buffer Composition: The reaction buffer must maintain the stability and activity of the polymerase while supporting multiple primer and probe interactions in the same tube.
- Instrumentation:
- Detection Capability: Ensure that the qPCR instrument can differentiate and quantify the specific fluorescent signals emitted by the dyes used in the multiplex setup. Instruments must have the appropriate filters and calibration settings to detect multiple colors.
- Validation and Verification:
- Cross-reactivity Tests: It’s critical to test the multiplex qPCR setup for cross-reactivity among the primer and probe sets to avoid non-specific amplifications.
- Efficiency and Sensitivity Tests: Validate the efficiency and sensitivity of the assay for each target, ensuring that the multiplexing does not compromise the assay’s overall performance.
Multiplexing in qPCR is a powerful method that offers significant benefits in terms of efficiency, cost, and data output. However, the complexity of assay design and the need for rigorous optimization and validation should not be underestimated. Properly designed multiplex qPCR assays can provide reliable and robust results, making them indispensable in fields requiring simultaneous detection and quantification of multiple genetic targets.
Thermal Profile Optimization in qPCR
In multiplex qPCR, thermal profile optimization is a critical step in ensuring that all primer sets used in the reaction function efficiently and effectively. This process involves designing and adjusting the thermal cycling conditions to accommodate the annealing temperatures of multiple primers, which can present unique challenges. Here’s a detailed look at how to optimize the thermal profile for multiplex qPCR and ensure reliable amplification of all targets.
Why Thermal Profile Optimization is Necessary
- Annealing Temperature: Each primer pair has an optimal annealing temperature where it binds specifically to its target sequence, avoiding non-specific binding or mismatches. In multiplex qPCR, where multiple primer pairs are used, ensuring that all primers anneal efficiently at the same temperature is crucial for balanced amplification.
- Amplification Efficiency: The efficiency of each amplification cycle depends on the ability of primers to bind to their targets and initiate DNA synthesis. If the thermal cycling conditions do not suit all primer pairs, some targets may be under-amplified or over-amplified, leading to inaccurate quantification.
- Specificity: A well-optimized thermal profile helps minimize non-specific amplifications, such as primer-dimer formations or off-target amplifications, ensuring that the results accurately reflect the specific targets.
Steps for Thermal Profile Optimization
- Initial Assessment: Start by assessing the melting temperatures (Tm) of each primer pair. The Tm provides a starting point for determining the annealing temperature for the qPCR cycle. Ideally, all primer pairs should have Tm values that are close to each other, within a 2°C to 3°C range.
- Gradient PCR: Conduct a gradient PCR experiment to identify an annealing temperature that works efficiently for all primer sets. This involves setting up multiple reactions with different annealing temperatures across a defined range, typically spanning 5°C to 10°C.
- Analyzing Results: Evaluate the efficiency of each reaction by examining the amplification curves or running an agarose gel to assess the yield and specificity of each target. Select the annealing temperature that provides balanced amplification across all targets.
- Adjusting Other Conditions: After identifying a suitable annealing temperature, optimize other thermal cycling conditions:
- Denaturation Temperature: Set the denaturation temperature high enough to ensure complete separation of the DNA strands without degrading the template or affecting the primers.
- Extension Temperature and Time: Ensure that the extension temperature and duration are suitable for the DNA polymerase being used, allowing for complete synthesis of the amplicons.
- Revalidation: After finalizing the thermal profile, revalidate it by performing a few test runs to ensure consistent amplification of all targets across multiple reactions.
Additional Considerations
- Reaction Buffer: Ensure that the buffer composition supports stable primer and polymerase interactions across the entire thermal range, preventing enzyme degradation or loss of primer activity.
- Multiplex Assay Complexity: The complexity of the assay can impact thermal profile optimization. The more targets included in a multiplex assay, the more challenging it may be to find a thermal profile that works for all.
- Instrument Calibration: Ensure that the qPCR instrument is properly calibrated to maintain the thermal cycling conditions accurately, which is essential for reproducibility and consistent results.
Thermal profile optimization is essential for balanced and specific amplification in multiplex qPCR. By carefully assessing and adjusting the annealing temperatures and other cycling conditions, researchers can achieve efficient amplification of all targets in a single reaction. This optimization not only improves the accuracy of the assay but also enhances its overall utility, making multiplex qPCR a powerful tool for genetic analysis in both research and diagnostic contexts.
Data Analysis and Interpretation in qPCR
Analyzing and interpreting data from quantitative PCR (qPCR) experiments involves understanding amplification curves and calculating relative gene expression accurately. This process is crucial for deriving meaningful biological conclusions from qPCR data. Here’s a comprehensive guide to the key steps and considerations in qPCR data analysis.
Understanding Amplification Curves
- Basics of Amplification Curves:
- Amplification curves in qPCR represent the accumulation of fluorescent signal during the PCR cycles. Each curve corresponds to a specific primer-probe set targeting a gene of interest or a control.
- The fluorescence intensity increases as the DNA template is amplified, typically shown as a sigmoidal (S-shaped) curve on a graph plotting fluorescence against the cycle number.
- Threshold and Baseline:
- Threshold Line: A horizontal line on the amplification plot that intersects the exponential phase of the amplification curves. The cycle at which the amplification curve crosses this threshold is termed the threshold cycle (Ct).
- Baseline: The initial cycles of the qPCR where little to no amplification is detected. This part is used to establish a baseline level of fluorescence to help distinguish between the background noise and the true amplification signal.
- Interpreting Curves:
- Single, Sharp Peak: Indicates specific amplification.
- Multiple Peaks or High Baseline: May indicate non-specific amplifications, such as primer-dimers or contamination.
- Efficiency: Can be assessed by the steepness of the curve; steeper curves generally indicate more efficient amplification.
Calculating Relative Gene Expression
- ΔCt Method:
- Calculate the difference in the Ct values between the target gene and an internal reference gene (housekeeping gene) for the same sample: ΔCt = Ct(target) – Ct(reference).
- This method assumes that the reference gene is stably expressed across all samples being compared.
- ΔΔCt Method (Comparative Ct Method):
- Used to compare gene expression levels across different samples or treatment groups.
- First, calculate the ΔCt for each sample as described above.
- Select one of the samples as a calibrator (often a control or untreated sample). Calculate ΔΔCt by subtracting the ΔCt of the calibrator from the ΔCt of each test sample: ΔΔCt = ΔCt(sample) – ΔCt(calibrator).
- Relative gene expression is then calculated as 2^(−ΔΔCt), which gives the fold change in gene expression relative to the calibrator.
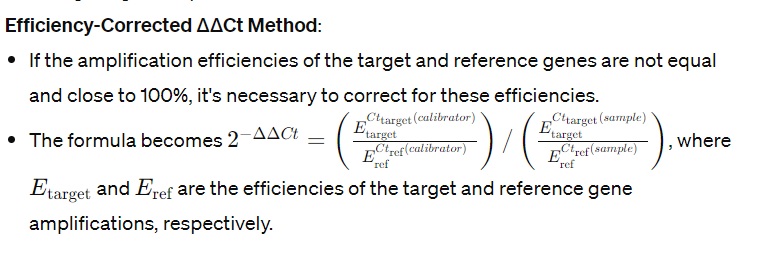
Considerations for Accurate Interpretation
- Selection of Reference Genes: Choose reference genes that are proven to be stably expressed across all experimental conditions and samples. Inappropriate reference genes can lead to significant errors in quantification.
- Validation of Assay: Run validation experiments to determine the amplification efficiency of each primer pair. Ideally, efficiencies should be between 90% and 110%.
- Reproducibility: Include technical replicates for each sample to ensure the reproducibility of the results.
- Quality Control: Regularly evaluate the specificity of the primer and probe sets using melting curve analysis or gel electrophoresis to confirm that only the specific target is being amplified.
Effective data analysis and interpretation in qPCR are pivotal for assessing gene expression accurately. By understanding amplification curves and applying appropriate calculation methods for relative gene expression, researchers can obtain reliable and insightful results that advance our understanding of genetic regulation and expression in diverse biological contexts.
Optimization Strategies for qPCR
Optimizing reaction conditions in quantitative PCR (qPCR) is crucial for enhancing the efficiency, sensitivity, and reliability of your results. Here, we explore practical tips for optimizing these conditions and address common troubleshooting issues along with their solutions.
Tips for Optimizing Reaction Conditions
- Primer and Probe Design:
- Ensure primers and probes are designed to specifically target the region of interest without forming dimers or secondary structures. Use software tools for checking specificity and potential interactions.
- Optimize primer and probe concentrations. Typically, primer concentrations range from 100 to 500 nM, and probe concentrations are around 100 to 250 nM, but these may need adjustment based on specific assay requirements.
- Template Quality and Quantity:
- Use high-quality, pure DNA/RNA as the template. Assess the purity by spectrophotometry (A260/A280 ratio) and check for degradation or contamination.
- Optimize the amount of template used. Too little may yield poor amplification, while too much can inhibit the reaction.
- Annealing Temperature:
- Optimize the annealing temperature by running a gradient PCR to find the optimal temperature that provides the best balance between specificity and yield.
- Enzyme Selection:
- Choose a high-quality, suitable DNA polymerase. For example, use a hot-start DNA polymerase to reduce non-specific amplification and primer-dimer formation.
- Magnesium and Buffer Conditions:
- Optimize MgCl2 concentration, which is crucial for enzyme activity and DNA denaturation. Typical concentrations range from 1.5 to 3.0 mM.
- Ensure the buffer is appropriate for the specific polymerase and reaction setup, maintaining the correct pH and ionic strength.
- Cycle Number:
- Determine the optimal number of cycles. Too many cycles can lead to plateau effects and increase background noise, while too few may result in undetectable signals.
Common Troubleshooting Issues and Solutions
- Non-specific Amplification or Primer-Dimers:
- Increase the annealing temperature to reduce non-specific binding.
- Adjust primer concentrations downward or redesign primers.
- Include a hot-start DNA polymerase to minimize activity at room temperature.
- Low Amplification Efficiency:
- Verify the quality and quantity of the template. Consider re-purifying or quantifying the template accurately.
- Adjust the enzyme and magnesium concentrations.
- Ensure that the thermal cycler is calibrated and functioning properly.
- High Background or Noise:
- Check for contamination in reagents or equipment. Use nuclease-free tubes and tips.
- Reduce the starting template amount if too much template is causing non-specific amplification.
- Optimize the probe and primer concentrations to reduce excess.
- Plateau Effect Too Early:
- Increase the amount of enzyme or improve buffer conditions to extend the efficiency of the reaction.
- Examine the possibility of reagent depletion; increasing the volume or concentration of dNTPs and MgCl2 might help.
- Fluctuating Ct Values Across Replicates:
- Ensure consistent sample preparation and pipetting techniques.
- Use precise and calibrated pipettes. Consider including more replicates to account for variability.
Conclusion
Optimizing qPCR reaction conditions involves a systematic approach to adjusting various components and settings based on the specific needs of your assay. Regularly revisiting and refining these conditions can significantly improve the performance of your qPCR assays. By addressing common issues effectively, you can achieve more reliable and reproducible results, which are essential for high-quality data in research and diagnostic applications.
Applications of qPCR
Quantitative PCR (qPCR) is a versatile and powerful technique that has revolutionized molecular biology and diagnostics due to its high sensitivity, specificity, and quantitative accuracy. It is employed across a wide range of scientific disciplines and industries. Below, we explore some of the key applications of qPCR in genetics, infectious disease testing, and environmental monitoring, illustrating its broad utility and impact.
Applications in Genetics
- Gene Expression Analysis:
- qPCR is routinely used to quantify changes in gene expression levels in cells or tissues under various conditions. This application is crucial for understanding gene function, identifying disease mechanisms, and evaluating the effects of treatments in research and clinical settings.
- Genotyping and Mutation Detection:
- qPCR enables rapid and accurate genotyping of individuals, helping identify genetic variants and mutations. This capability is vital for personalized medicine, where genetic information guides treatment decisions, and for population genetics studies.
- Cancer Research:
- In oncology, qPCR assesses tumor genetics and gene expression profiles, helping identify biomarkers for cancer diagnosis and prognosis. It also measures minimal residual disease and monitors the effectiveness of therapy by detecting cancer-specific genetic changes.
Applications in Infectious Disease Testing
- Pathogen Detection:
- qPCR is extensively used in clinical microbiology to detect and quantify pathogenic bacteria, viruses, and fungi with high precision. It is essential for diagnosing infections quickly and accurately, such as influenza, COVID-19, and HIV.
- Antimicrobial Resistance Testing:
- By detecting genes associated with resistance, qPCR helps determine the susceptibility of microbes to antibiotics, guiding effective treatment strategies and helping manage antibiotic resistance globally.
- Viral Load Quantification:
- For viral infections, qPCR quantifies the amount of viral RNA or DNA in a patient’s samples, providing crucial information for diagnosing, monitoring, and managing infectious diseases such as hepatitis and HIV.
Applications in Environmental Monitoring
- Water Quality Testing:
- qPCR is used to assess water quality by detecting and quantifying microbial contaminants, including pathogens and toxin-producing algae. This application is crucial for public health and environmental protection.
- Soil and Biodiversity Assessment:
- In environmental science, qPCR aids in evaluating soil health and biodiversity by detecting and quantifying specific organisms and microbes, contributing to ecosystem assessments and conservation efforts.
- Food Safety:
- qPCR tests for the presence of pathogens, GMOs, and allergens in food products, ensuring compliance with safety standards and regulations to protect consumer health.
The applications of qPCR are vast and impactful, extending beyond the few examples listed here. Its ability to provide rapid, sensitive, and accurate quantitative results makes qPCR indispensable in modern science and medicine. Whether it’s advancing our understanding of genetic processes, improving the diagnosis and treatment of diseases, or ensuring the safety of our environment and food supply, qPCR continues to be a cornerstone technology in research and diagnostics. Its ongoing development and integration into various fields promise even broader applications and innovations in the future.
Conclusion: The Impact and Future of qPCR Technology
Quantitative PCR (qPCR) stands as one of the most influential advancements in molecular biology, offering unmatched versatility and precision in the detection and quantification of nucleic acids. As we have explored, its applications span across diverse fields including genetics, infectious disease diagnosis, environmental monitoring, and beyond, making it an indispensable tool in both research and clinical settings.
Recap of qPCR’s Versatility and Precision
- Versatility: qPCR’s ability to quantify DNA and RNA from various sources underlies its vast range of applications. From basic research in genetics to critical uses in disease diagnosis and environmental assessments, qPCR adapts to meet diverse scientific needs.
- Precision: The technique’s high sensitivity allows for the detection of minute amounts of genetic material, providing accurate and reproducible data. This precision is crucial for making informed decisions in healthcare, such as determining viral loads in patients or detecting early signs of genetic disorders.
Encouragement for Further Exploration
- Advancements in qPCR: As technology progresses, we anticipate further enhancements in qPCR methodologies that will expand its utility. Innovations may include improved enzyme efficiency, enhanced multiplexing capabilities, and integration with digital and droplet technologies, which promise even lower detection limits and higher throughput.
- Educational and Research Opportunities: Scientists, clinicians, and students are encouraged to delve into the rich potential of qPCR. Whether through academic studies, laboratory research, or clinical practice, engaging with this technology can lead to significant contributions to science and medicine.
- Collaborative Endeavors: The ongoing development of qPCR benefits greatly from interdisciplinary collaborations. Combining efforts across fields such as bioinformatics, chemistry, and engineering can lead to novel applications and optimizations of qPCR that could revolutionize how we understand and manipulate genetic information.
qPCR technology continues to evolve, pushing the boundaries of what is possible in scientific research and medical diagnostics. Its ability to provide rapid, sensitive, and precise genetic analysis is more than just a laboratory convenience; it is a transformational force across multiple disciplines. As we continue to uncover and harness the capabilities of qPCR, the future holds promising advancements that will undoubtedly enhance our ability to decode the complexities of biology and improve human health globally. Embracing these opportunities, continuing to innovate, and expanding the applications of qPCR will be key to unlocking even more profound insights and achievements in the years to come.